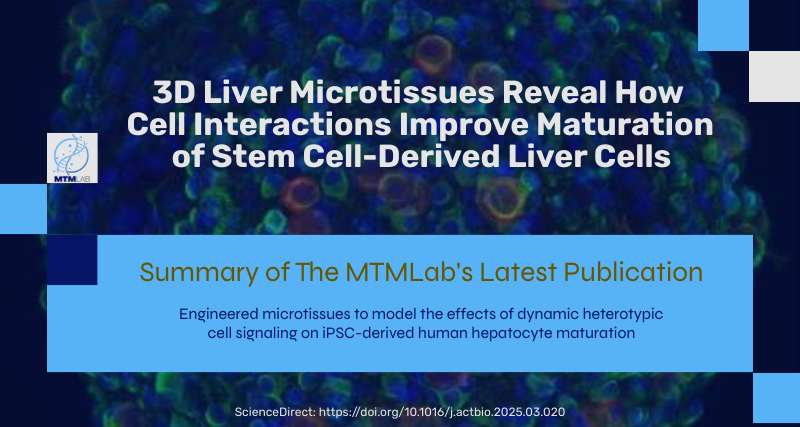
Our latest study addresses a critical challenge in liver tissue engineering: stem cell-derived liver cells (iHeps) typically remain functionally immature, limiting their usefulness for drug testing and disease modeling. Our research team created 3D microtissues using droplet microfluidics technology by: • Encapsulating iHeps in tiny collagen gel droplets (~250 μm diameter) • Coating these structures with various non-parenchymal cells (NPCs) • Testing different combinations and sequences of supporting cells Key findings: 1) Embryonic fibroblasts and liver sinusoidal endothelial cells (LSECs) produced the most mature iHeps compared to other tested cell types 2) Sequential application of cell signals (embryonic fibroblasts first, then LSECs) yielded optimal maturation 3) Specific growth factors like stromal-derived factor-1 alpha were identified as important maturation enhancers 4) Gene expression analysis confirmed that LSEC/iHep microtissues closely resembled adult human liver cells This platform enables researchers to identify critical cellular interactions and molecular signals that drive liver cell maturation, providing valuable insights for developing more physiologically relevant liver models for drug screening and regenerative medicine applications. https://www.sciencedirect.com/science/article/pii/S174270612500193X SIMPLE SUMMARY: Embryonic fibroblasts and liver sinusoidal endothelial cells dramatically improved iHep maturation compared to other cell types tested, producing more functionally mature liver cells. Sequential application proved crucial—adding embryonic fibroblasts first, followed by endothelial cells, yielded optimal maturation. Specific growth factors including stromal-derived factor-1 enhanced this process. This research enables creation of more authentic mini-liver tissues that function like human liver. These improved models support better drug testing, disease research, and regenerative medicine applications.
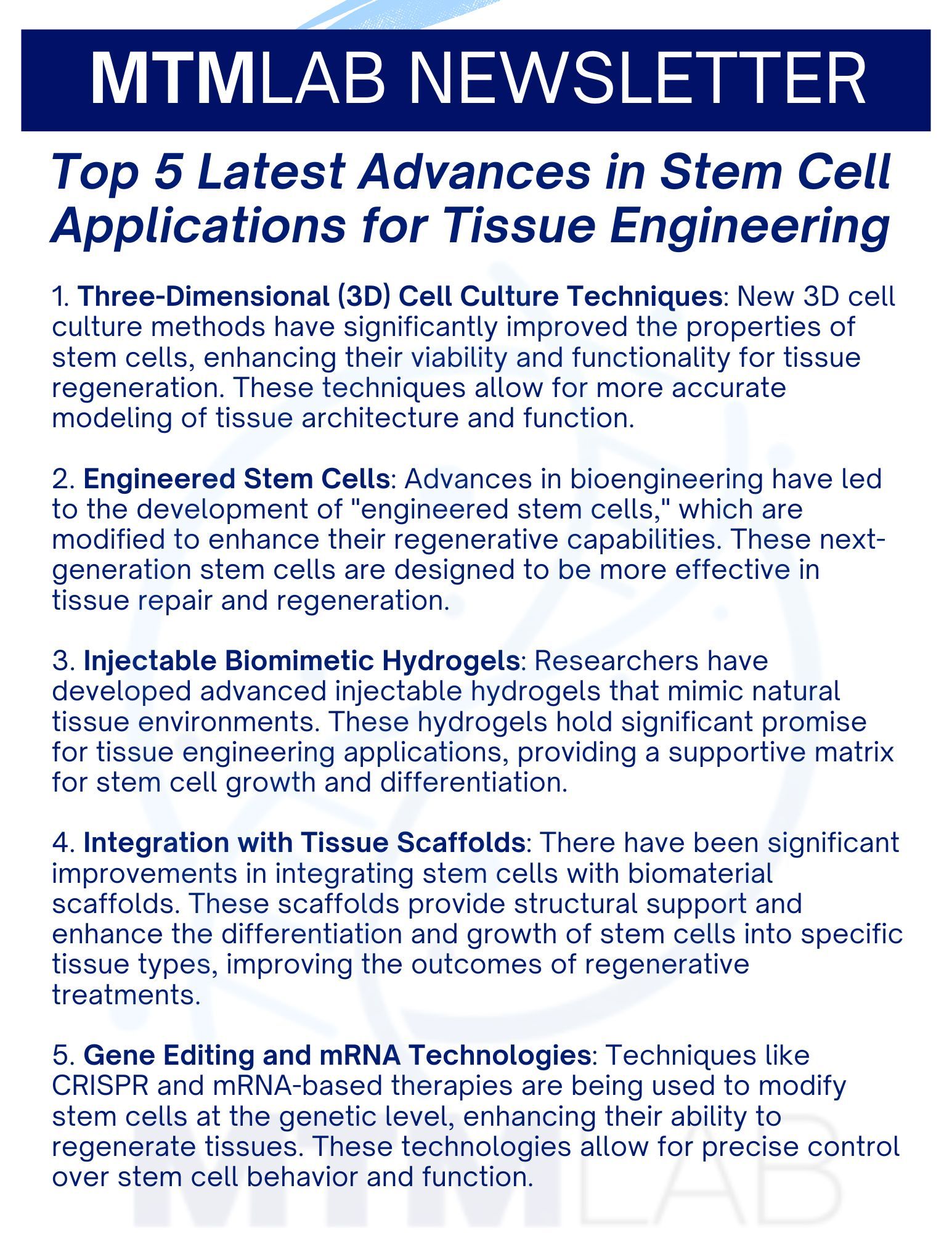
1. Three-Dimensional (3D) Cell Culture Techniques : New 3D cell culture methods have significantly improved the properties of stem cells, enhancing their viability and functionality for tissue regeneration. These techniques allow for more accurate modeling of tissue architecture and function. 2. Engineered Stem Cells : Advances in bioengineering have led to the development of "engineered stem cells," which are modified to enhance their regenerative capabilities. These next-generation stem cells are designed to be more effective in tissue repair and regeneration. 3. Injectable Biomimetic Hydrogels : Researchers have developed advanced injectable hydrogels that mimic natural tissue environments. These hydrogels hold significant promise for tissue engineering applications, providing a supportive matrix for stem cell growth and differentiation. 4. Integration with Tissue Scaffolds : There have been significant improvements in integrating stem cells with biomaterial scaffolds. These scaffolds provide structural support and enhance the differentiation and growth of stem cells into specific tissue types, improving the outcomes of regenerative treatments. 5. Gene Editing and mRNA Technologies : Techniques like CRISPR and mRNA-based therapies are being used to modify stem cells at the genetic level, enhancing their ability to regenerate tissues. These technologies allow for precise control over stem cell behavior and function.
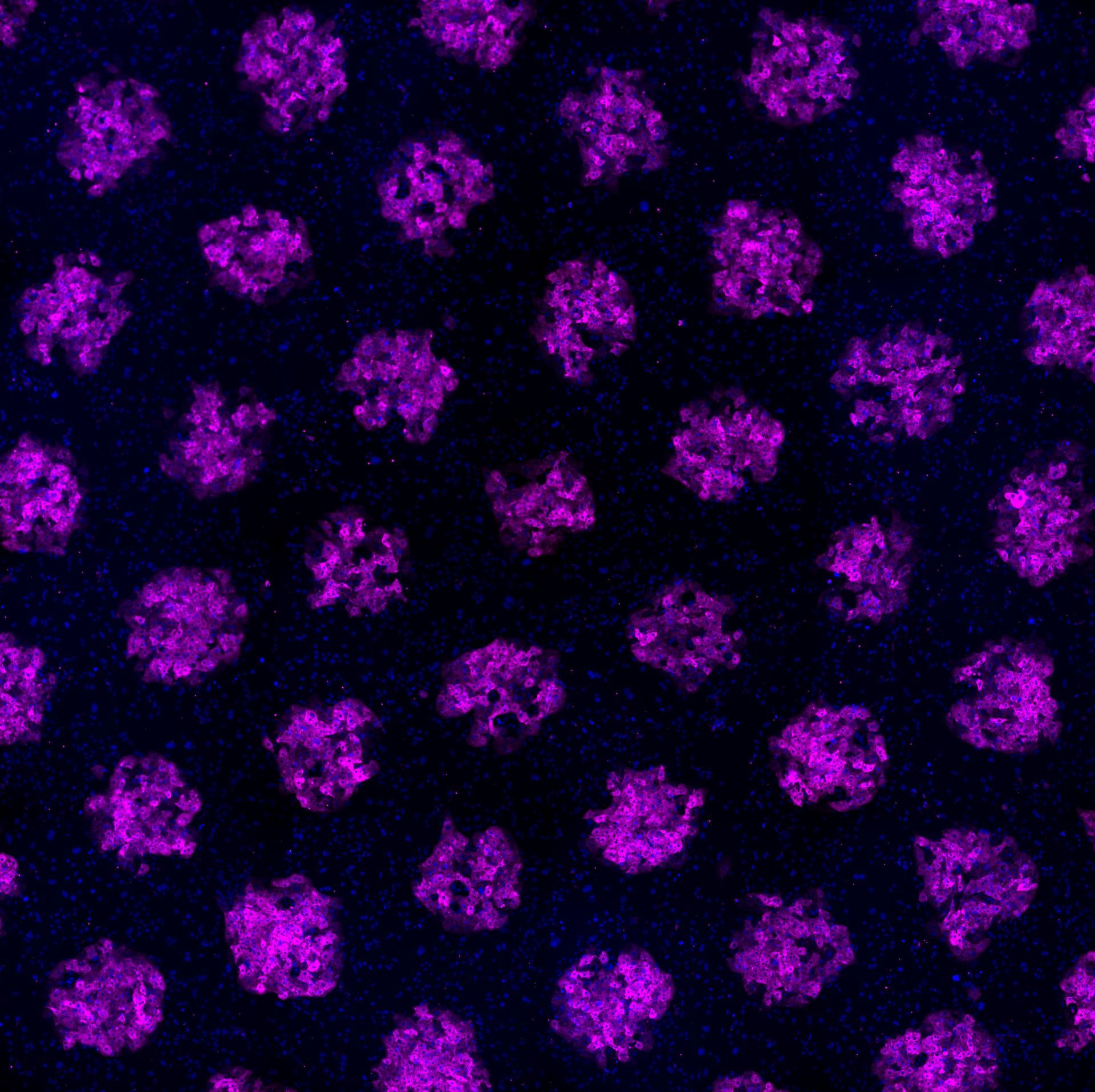
2024 The MTM Lab has been awarded an NIDDK R01 (National Institute of Diabetes and Digestive and Kidney Diseases) grant to develop a novel microfluidic approach to elucidate the effects of soluble factor gradients, individually and in controlled combinations, on zonated functions in primary liver cells from rodents and humans towards determining species-specific effects . Ultimately, our novel devices can be used to investigate the mechanisms underlying liver zonation, chemical-induced zonated hepatotoxicity, and how zonation is perturbed in liver diseases, such as non-alcoholic fatty liver disease and hepatocellular carcinoma. The MTM Lab has been awarded a NIEHS (National Institute of Environmental Health Sciences) grant to develop a high throughput system to test placental cell invasion using a 3D placental microtissue coupled with hepatic liver biotransformation . This first-of-its-kind hepatic-placenta organ-tandem on a chip will simulate the liver metabolism that chemicals undergo in vivo prior to reaching the placental bed. This state-of-the-art in vitro platform will be the first step towards incorporating organism-level organization into reproductive risk assessment using a non-animal-based approach. The MTM Lab has been awarded a NIEHS (National Institute of Environmental Health Sciences) grant to develop a human gut-liver platform with microbiome interactions for in vitro toxicology . These first-of-its-kind scalable human gut-liver models will be developed for in vitro applications, such as compound screening and disease modeling, and be used to elucidate the effects of reciprocal tissue crosstalk on cell phenotype modulation. 2023 The MTM Lab has been awarded a NIDDK (National Institute of Diabetes and Digestive and Kidney Diseases) grant to analyze the synergistic effects of extracellular matrix composition and stiffness, multicellular interactions, and soluble triggers of NAFLD in cellular phenotypic alterations , which could aid the development of novel drug therapies for this disease. The MTM Lab has been awarded a NIAAA (National Institute on Alcohol Abuse and Alcoholism) grant to develop a first-of-its-kind organotypic mouse liver model and investigate the effects of alcohol on multiple liver cell types in this model with comparisons to an in vivo mouse model of ALD that recapitulates several key features of human ALD. This platform can aid in understanding the molecular mechanisms underlying alcohol-associated liver disease.
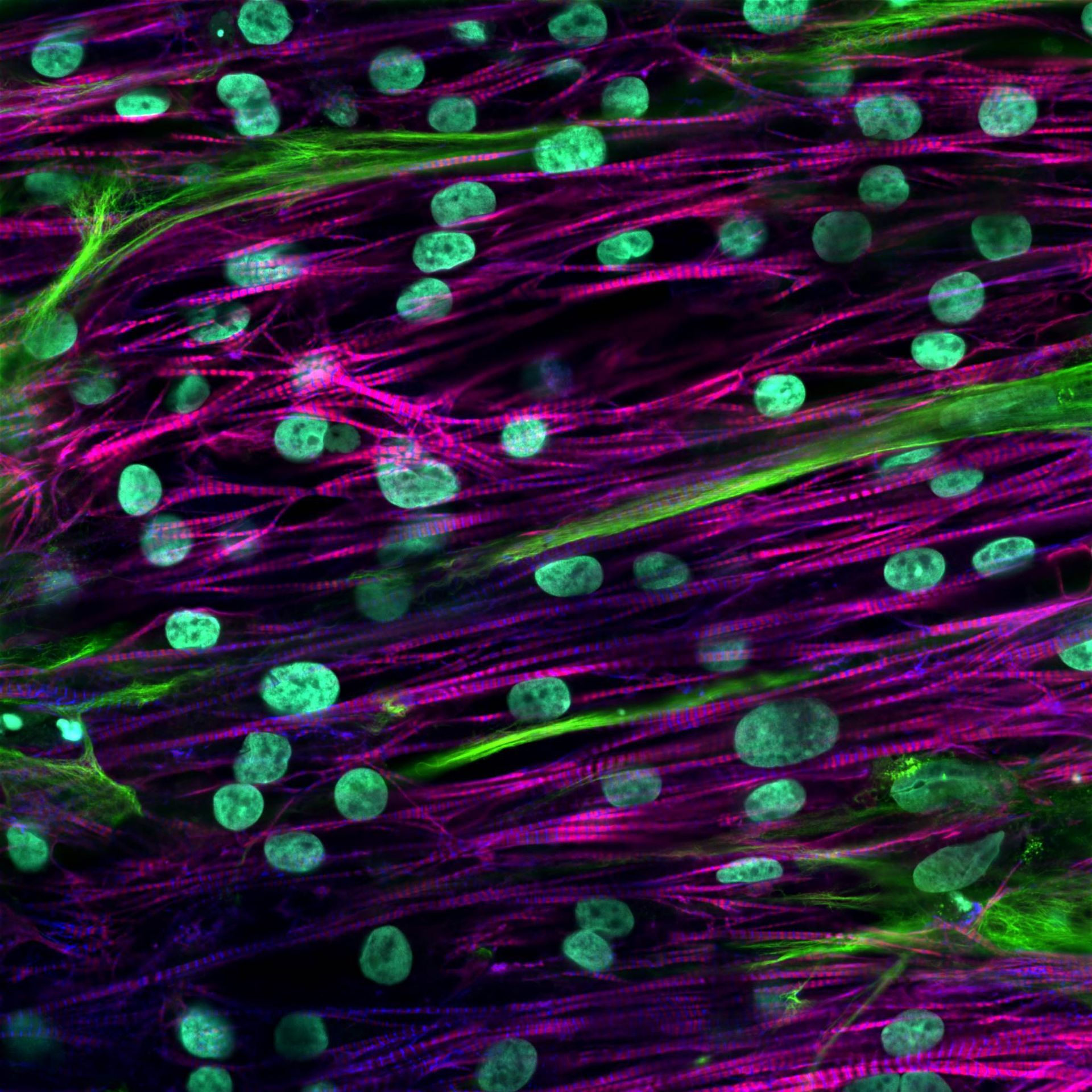
Students in the MTM lab presented 12 abstracts at the 2023 Annual Meeting of the Biomedical Engineering Society in Seattle, WA. Students in the MTM lab presented 11 abstracts in the form of 7 platform talks and 4 posters at the 2021 Annual Meeting of the Biomedical Engineering Society in Orlando, FL. Regeant Panday, a PhD student in the MTM lab, presented his work with 3D human liver tissues (poster) at the MicroTAS 2020 conference. The MTM lab presented 6 accepted abstracts (2 talks and 3 posters) at the Annual Meeting of the Biomedical Engineering Society. The MTM lab presented 8 abstracts, one as an oral presentation and seven as poster presentations at The Second Annual UIC Bioengineering Research Symposium. Congratulations to Grace Brown, Hardik Dabas, Demi Ibrahim, David Kukla, Jennifer Liu, Chase Monckton, Regeant Panday, and Yang Yuan for these presentations. The MTM lab presented 3 posters at the biannual meeting of the Center for Advanced Design and Manufacturing of Integrated Microfluidics (CADMIM) in Irvine, CA. Congratulations to Jennifer Liu, Grace Brown, and David Kukla for these presentations. The MTM lab presented 8 abstracts at the annual meeting of the Biomedical Engineering Society (BMES), incluidng 3 oral presentations and 5 poster presentations. Congratulations to Grace Brown, David Kukla, Jennifer Liu, and Chase Monckton for these presentations. Dr. Khetani presented MTM lab's research on a microfluidic human liver model at the annual meeting of the Biomedical Engineering Society (BMES) in Phoenix, AZ. David Kukla , Matt Davidson and Dr. Khetani presented MTM research in the form of oral talks and poster presentations at the annual meeting of the Biomedical Engineering Society (BMES) in Minneapolis, MN.
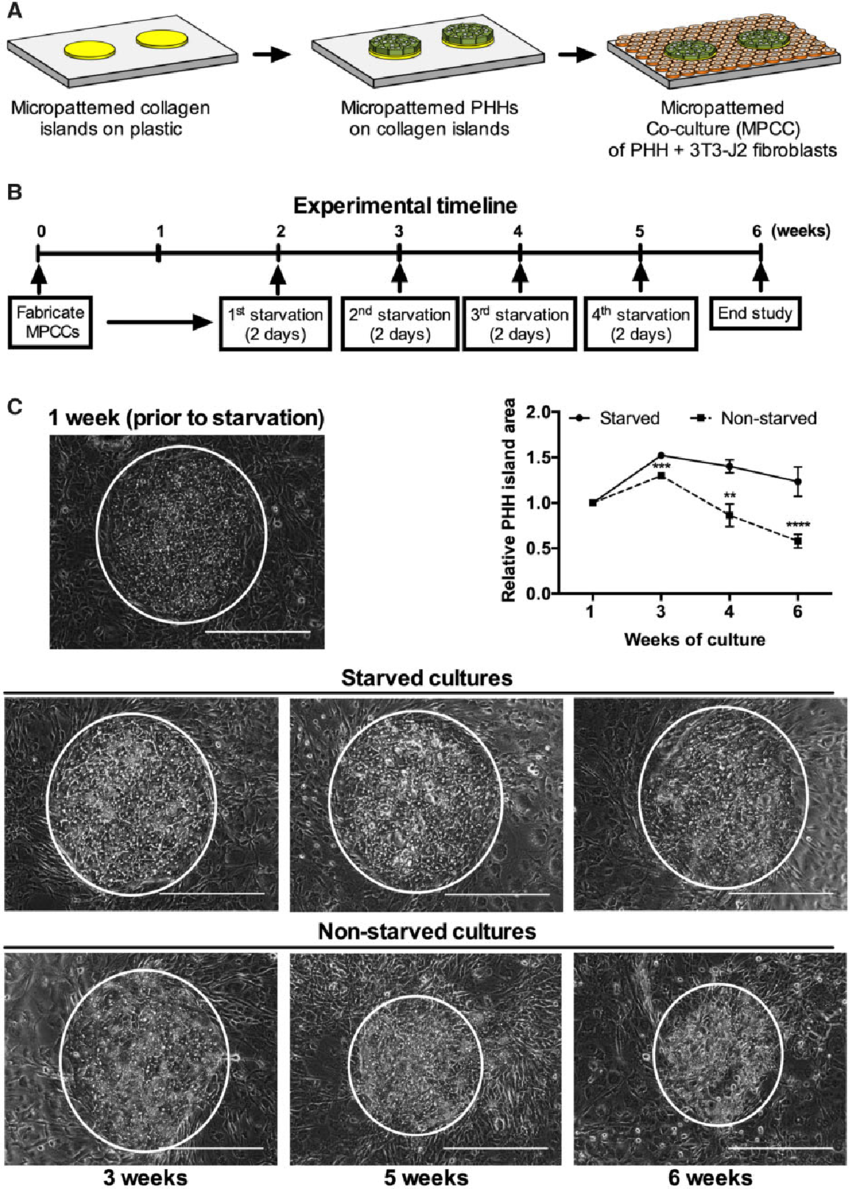
Primary human liver cells (PHHs) are crucial for testing harmful drug reactions in human patients. Normally, these cells lose their functions quickly in simple cultures, but when grown with other supportive cells, they last 2-4 weeks. However, since liver cells in the body function for much longer (200-400 days), we want to extend their functional life in the lab to study long-term drug effects and diseases. Fasting has been shown to improve the health & longevity of tissues like the liver. We thought that mimicking fasting in cell cultures might activate beneficial pathways and extend the life of PHHs. To do this, we periodically removed serum and hormones from a 'micropatterned coculture' of liver cells, which is a special arrangement of liver cells surrounded by connective tissue cells (fibroblasts) for support. A weekly 2-day starvation period extended the life of PHHs to over 6 weeks, compared to just 3 weeks without fasting. This fasting also improved the function of simpler 'monoculture' liver cells for 2 weeks. In the 'micropatterned cocultures', fasting activated a key energy-regulating protein called AMPK and prevented the supportive fibroblast cells from overgrowing, helping maintain liver cell structure (polarity). Similar benefits were seen when we activated AMPK with a drug called metformin or stopped the growth of supportive cells with another drug, mitomycin-C. Finally, liver cells in the fasting cultures were better at predicting drug toxicity and had higher drug-processing activities even after 5 weeks. In summary, intermittent fasting in cell cultures extends the functional life of liver cells, making them more useful for drug testing. Publication >> Intermittent Starvation Extends the Functional Lifetime of Primary Human Hepatocyte Cultures
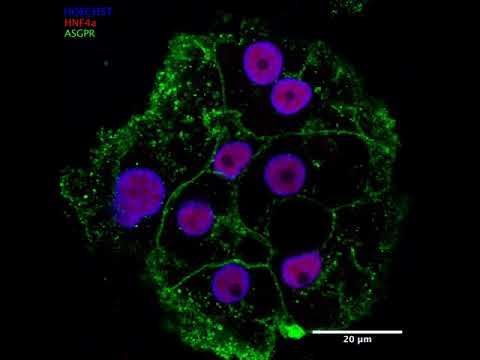
iPSC-derived hepatocytes are liver cells created from induced pluripotent stem cells (iPSCs). iPSCs are special because they can be generated from adult cells—like skin or blood cells—through a process called reprogramming. This involves introducing certain genes that revert these adult cells back to a pluripotent state, meaning they can turn into almost any cell type in the body. Once iPSCs are established, scientists can guide them to become hepatocytes, the main cells that make the liver function. These iPSC-derived hepatocytes are incredibly useful for a range of purposes: Disease Modeling: Researchers can create iPSCs from a patient’s own cells and turn them into hepatocytes to study liver diseases on a cellular level. Drug Testing and Toxicity Screening: These liver cells offer a human-based model to test how new drugs work and whether they’re safe, potentially reducing the need for animal testing. Regenerative Medicine: iPSC-derived hepatocytes could be key to developing cell-based therapies for liver diseases or even creating bioengineered liver tissue. Basic Research: By studying these cells, scientists can gain deeper insights into liver development, function, and the underlying mechanisms of liver diseases. In short, iPSC-derived hepatocytes are a powerful tool in biomedical research and therapeutic development, offering a personalized, human-relevant way to explore liver function and disease. Related MTMLab Publication: https://pubmed.ncbi.nlm.nih.gov/39082962/
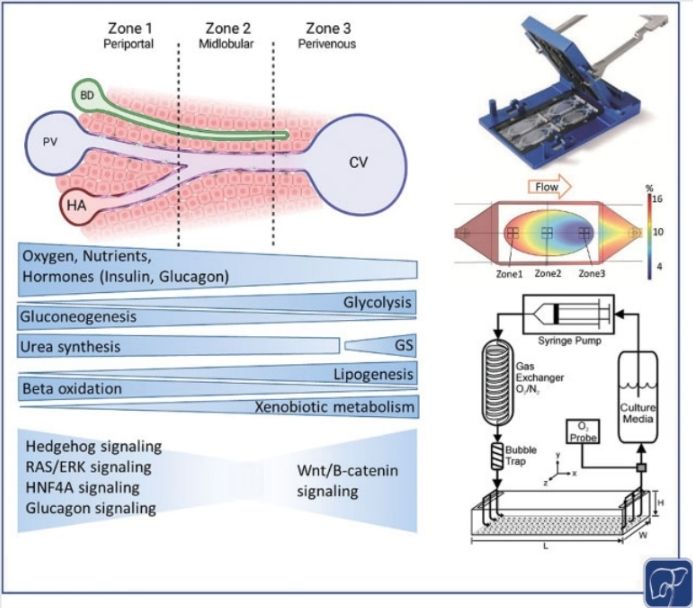
Liver zonation is a BIG topic, highly important for helping us develop 'liver tissue surrogates' (or liver mini models) for disease modeling and developing better therapeutics. In this comprehensive paper, our Lab explores liver zonation in depth with key findings, such as: how several liver diseases initiate in specific 'zones' of the liver how specialized plates and microfluidic devices are useful to investigate hepatic functions how oxygen, hormones and nutrients interact at a molecular level to regulate liver zonation how a deeper understanding of liver zonation can lead to better cell-based therapies Publication >> https://www.researchgate.net/publication/358376897_The_Role_of_Liver_Zonation_in_Physiology_Regeneration_and_Disease
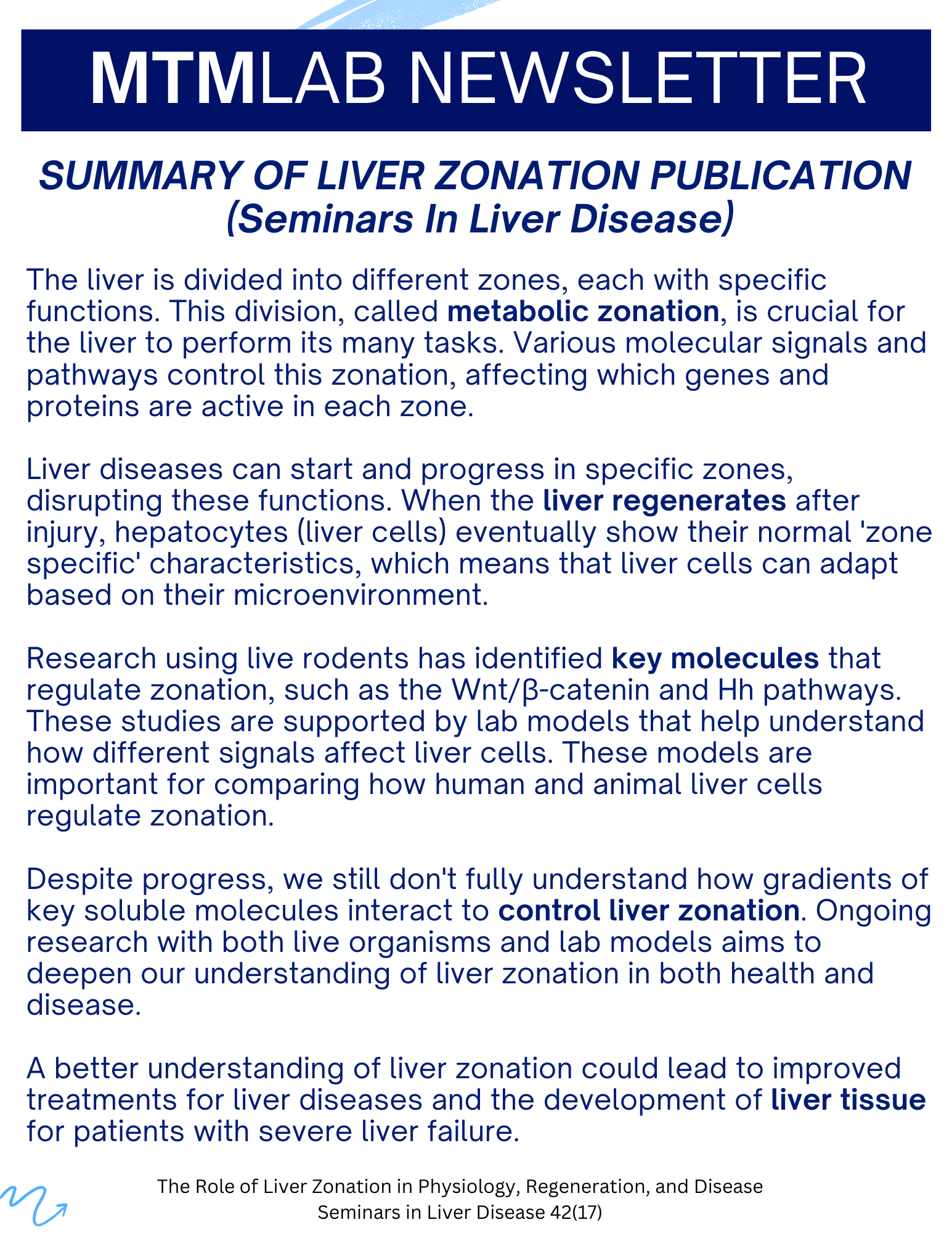
The liver is divided into different zones, each with specific functions. This division, called metabolic zonation, is crucial for the liver to perform its many tasks. Various molecular signals and pathways control this zonation, affecting which genes and proteins are active in each zone. Liver diseases can start and progress in specific zones, disrupting these functions. When the liver regenerates after injury, hepatocytes (liver cells) eventually show their normal 'zone specific' characteristics, which means that liver cells can adapt based on their microenvironment. Research using live rodents has identified key molecules that regulate zonation, such as the Wnt/β-catenin and Hh pathways. These studies are supported by lab models that help understand how different signals affect liver cells. These models are important for comparing how human and animal liver cells regulate zonation. Despite progress, we still don't fully understand how gradients of key soluble molecules interact to control liver zonation. Ongoing research with both live organisms and lab models aims to deepen our understanding of liver zonation in both health and disease. A better understanding of liver zonation could lead to improved treatments for liver diseases and the development of liver tissue for patients with severe liver failure. Publication >> https://www.researchgate.net/publication/358376897_The_Role_of_Liver_Zonation_in_Physiology_Regeneration_and_Disease
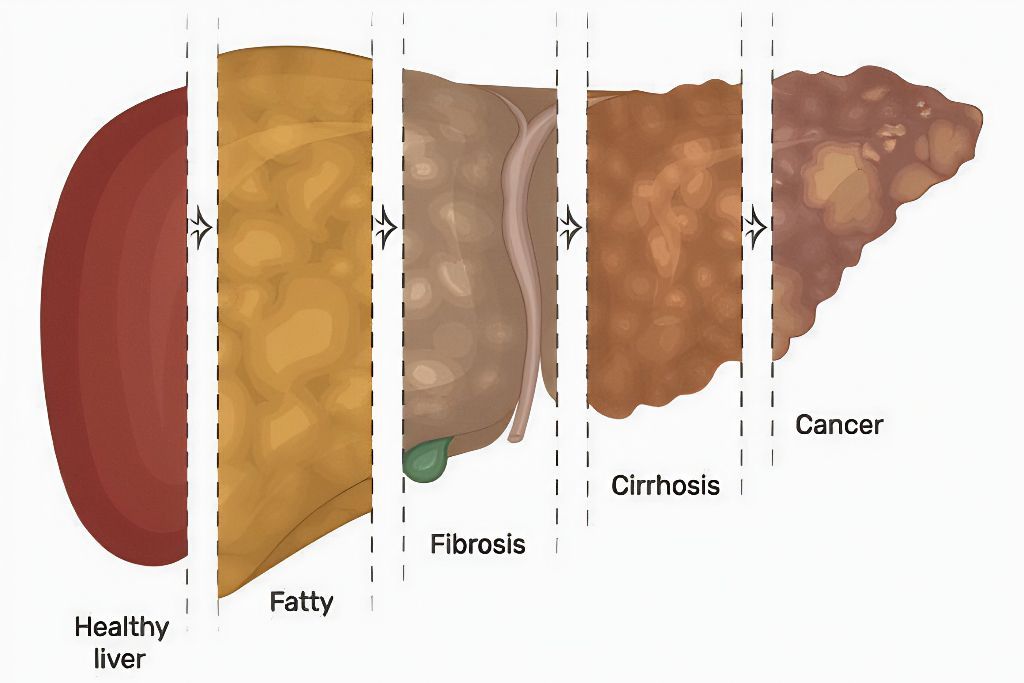
Understanding Alcoholic Fatty Liver Disease Alcoholic Fatty Liver Disease (AFLD) is a significant condition resulting from excessive alcohol consumption, leading to a spectrum of liver abnormalities ranging from simple steatosis to cirrhosis. This section outlines the critical aspects of AFLD, including its definition, epidemiological scope, contributing factors, and the progressive nature of the disease. Definition and Progression Alcoholic fatty liver disease is characterized by hepatic steatosis, where fat accumulates in the liver due to heavy alcohol use. When individuals consume more alcohol than the liver can process, it disrupts the breakdown of fats. If left unchecked, AFLD can advance from fatty liver to alcoholic hepatitis, an acute liver inflammation, and potentially to cirrhosis, where healthy liver tissue is substituted with scar tissue. Epidemiology and Prevalence The prevalence of AFLD is closely tied to patterns of alcohol consumption globally. Notably, it is more common in populations with higher rates of heavy alcohol use and binge drinking. Among those who consume alcohol excessively, AFLD is often the earliest form of alcohol-related liver disease (ALD) encountered. Risk Factors and Alcohol Consumption Major risk factors for developing AFLD include: Heavy Alcohol Use: Consistent intake of large quantities of alcohol. Binge Drinking: Consuming four to five drinks within two hours. Metabolic Syndrome: A cluster of conditions such as obesity, diabetes, and hypertension. High Body Mass Index (BMI): Increased body fat is associated with greater risk of fatty liver disease. These risk factors amplify the likelihood of developing AFLD and further progression to severe liver disease. Stages of Liver Disease The stages of liver disease due to alcohol use evolve over time: Steatosis (Fatty Liver): Fat accumulation in liver cells, often asymptomatic. Alcoholic Hepatitis: Inflammation and cell death occur in the liver. Fibrosis: Excess fibrous tissue builds up in the liver due to ongoing inflammation. Cirrhosis: Final stage, with widespread fibrosis and impaired liver function, which can be categorized as: Compensated Cirrhosis: The liver still functions despite damage. Decompensated Cirrhosis: Liver function is inadequate, leading to serious health complications. Each stage marks a progression in the severity of AFLD and potential for recovery decreases as the disease advances. Diagnosis and Treatment Strategies Effective management of Alcoholic Fatty Liver Disease (AFLD) involves prompt diagnosis and the implementation of treatment strategies aimed at mitigating liver damage and managing symptoms. AFLD treatment includes lifestyle modifications, medications, and in severe cases, liver transplantation. Recognizing Symptoms and Complications Patients with AFLD may experience a range of symptoms including fatigue, jaundice (yellowing of the skin and eyes), and ascites (accumulation of fluid in the abdomen). Complications such as portal hypertension, which can lead to gastrointestinal bleeding, and hepatic encephalopathy, characterized by mental confusion, are indicative of disease progression and warrant immediate medical attention. Diagnostic Testing and Assessment Diagnosis begins with an assessment of liver function through blood tests to evaluate liver enzymes, bilirubin levels, and other relevant markers. Imaging tests, including ultrasound, MRI, and potentially liver biopsy, are employed to assess the extent of liver damage. A biopsy can confirm the diagnosis by revealing fatty infiltration, inflammation, and liver cell damage. Conventional and Emerging Treatments The cornerstone of AFLD treatment is the cessation of alcohol consumption to prevent further liver damage. In addition to promoting abstinence, addressing alcohol dependency through counseling or support groups may be necessary. Medicines such as corticosteroids or pentoxifylline are prescribed for severe cases. When liver damage is advanced, liver transplantation may be considered. Emerging treatments, such as Vitamin E supplementation, are being researched for their potential to improve liver health in AFLD patients. Lifestyle and Long-Term Management Managing alcoholic fatty liver disease requires a comprehensive approach that focuses on sustainable lifestyle changes to aid in recovery and prevent further liver injury. These changes include a combination of diet, exercise, and weight management strategies; alcohol abstinence with supportive networks; and ongoing monitoring to prevent additional damage to the liver. Diet, Exercise, and Weight Management A healthy diet and regular exercise are crucial for managing body weight and reducing liver fat. A balance of macronutrients and micronutrients supports liver health and helps correct malnutrition: Weight Loss: Gradual weight loss, through calorie restriction and increased physical activity, can reduce liver fat. Aim for a steady loss of 0.5 to 1 kg (1.1 to 2.2 lbs) per week. Healthy Diet: Incorporate a diet high in fruits, vegetables, whole grains, and lean proteins while low in saturated fats and refined sugars. Body Mass Index (BMI): Maintain a BMI within the healthy range to reduce the risk of liver damage. Regular Exercise: Engage in at least 150 minutes of moderate-intensity exercise or 75 minutes of vigorous exercise per week. Alcohol Abstinence and Support Systems Abstinence from alcohol is imperative for individuals with alcoholic fatty liver disease: Complete Abstinence: Avoiding all forms of alcohol can halt the progression of liver disease and is essential for recovery. Support Systems: Seek out support groups, counseling, or medical programs specialized in alcohol dependency to provide necessary encouragement and accountability. Monitoring and Preventing Further Injury Continued surveillance is key in managing alcoholic fatty liver disease and preventing complications: Liver Health Monitoring: Regular check-ups with a healthcare provider, including liver function tests, can detect signs of liver injury early. Preventative Measures: Vaccinations for hepatitis A and B, controlling cholesterol levels, and managing other health conditions like diabetes can minimize additional liver stress. Nutritional Support: In cases of malnourishment, seek advice from a nutritionist or dietician to ensure adequate nutritional intake without overburdening the liver.